Retinoids in the pancreas
Introduction
Retinoids (vitamin A and its analogs) must be obtained from the diet where they are found primarily as retinyl ester and retinol, and as provitamin A carotenoids, like β-carotene, that can be converted by mammals to retinoid (1). The body requires retinoids to maintain normal cell proliferation, differentiation, apoptosis, and other cellular functions (2,3). Aside from in vision, where 11-cis-retinaldehyde serves as the visual chromophore (4), most retinoid actions within the body involve transcriptional regulatory effects that are mediated primarily by all-trans-retinoic acid (5-8). These retinoic acid species are potent transcriptional regulators controlling expression of more than 500 genes (8). The transcriptional regulatory activity of retinoic acid is mediated by six distinct ligand-dependent transcription factors (5-7). These include three retinoic acid receptors (RAR-α, RAR-β and RAR-γ) and three retinoid X receptors (RXR-α, RXR-β, and RXR-γ) (5-7). Each of these transcription factors is encoded by separate genes and can exist in multiple protein forms that are generated through alternative splicing or usage of alternative transcription start sites (5-7). The RARs and RXRs are members of the steroid/thyroid/retinoid superfamily of nuclear hormone receptors and recognize specific response elements present within genes (5-7). There is also some evidence that all-trans-retinoic acid can bind peroxisome proliferator-activated receptor beta/delta (PPARβ/δ) and that this accounts for some retinoid actions within the body (9). Recently, there has been a growing understanding that retinoic acid can act in a non-genomic manner to regulate directly signaling networks within the cell (10).
To understand retinoid actions in the body, it is first necessary to understand that retinoid metabolism is highly specialized, involving a number of different chemical species (retinyl esters, retinol, retinaldehyde, and retinoic acid). This metabolism utilizes enzymes and extra- and intracellular retinoid-binding proteins that also are specific to retinoid metabolism. To provide the reader with some insight into the retinoid metabolism that is relevant to this review, we have included Figure 1 which provides a very simplified overview of this metabolism. We note that within the body retinoid metabolism, as described by the entirety of the literature, is exceeding complex, involving the actions of many different enzymes and many different intra- and extracellular retinoid-binding proteins. Our goal in presenting a simplified overview of these processes in Figure 1 is to provide the reader with readily accessible generalized information on these processes.
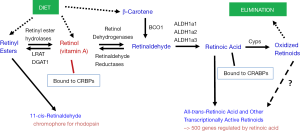
In this review we will first focus on the role of retinoids in the adult pancreas. Retinoids play an important role in energy metabolism and are required for maintaining the normal endocrine activities of the adult pancreas (11). We also will consider the role that retinoids play in the biology of pancreatic stellate cells (PSCs). Like hepatic stellate cells (HSCs) (12), the PSCs are characterized by large retinoid-containing lipid droplets that are present within the cytoplasm of these pancreatic cells (13-15). These two roles for retinoids within the adult pancreas, ones that require retinoic acid and RAR/RXR activity, will be the primary focus of this review. It also has long been known that retinoids are required by the pancreas to allow for normal pancreas development in utero. The literature regarding the role of retinoids in maintaining normal embryonic development is a very large one and we will only briefly review this literature to familiarize the reader with retinoid actions in the developing pancreas.
Role of retinoids in adult pancreatic islets and β-cells
Research interest in the possible importance of retinoids and retinoid signaling in pancreatic endocrine function in adults came of age in the mid-1980s. The early research reports in this area primarily involved the study of cell lines in culture and animal studies, predominantly involving rats, where the dietary content of retinoid provided to the animals was manipulated. More recent research has extended these approaches to studies of a number of genetically manipulated mouse models, allowing for more in depth understanding of retinoid actions in maintaining hormone secretion from the cells of the islets of Langerhans. We will summarize first the early studies aimed at understanding how retinoids might affect insulin and glucagon secretion by the endocrine pancreas. We will then consider the more detailed molecular studies reported in the last five years.
The early literature
The early work on retinoids and their effects on hormone production by cells of the islets was spearheaded by Chertow and colleagues, but other laboratories also contributed to this research. Initially, Chertow et al. studied the effects of 13-cis-retinoic acid on rat islet cell-to-cell adhesion and glucose stimulated insulin secretion (16). Treatment of the isolated rat islets with 0.1 or 1.0 μM 13-cis-retinoic acid caused islet cells to adhere more tightly to each other. At very high concentrations (100 μM) 13-cis-retinoic acid treatment stimulated insulin secretion at 9.7, 12.5, 16.7 and 27.7 mM glucose. Maximal effects of 13-cis-retinoic acid (174% of control) were observed during second phase insulin release at 9.7 mM glucose (16). It should be noted that this early study was carried out several years before all-trans- and 9-cis-retinoic acid had been identified as transcriptionally active retinoid species (2,3,5,6). Although 13-cis-retinoic acid has been used clinically to treat skin and other diseases, it is far less potent in regulating transcription than either its all-trans- or 9-cis-isomer (2,3,5,6).
Immunohistochemical studies of the rat pancreas localized plasma retinol-binding protein [RBP or RBP4; the sole specific transport protein for retinol in the blood (17,18)], cellular retinol-binding protein, type I (CRBPI) [an intracellular protein that binds retinol and facilitates its metabolic conversion to retinoic acid (19)] and transthyretin (TTR) [a plasma protein that binds RBP in the circulation preventing its renal filtration (17,18)] to the islets of Langerhans (20). Both RBP and CRBPI were peripherally distributed within the islets, with an anatomic distribution that resembles that of the glucagon-containing α-cells. Interestingly, immunoreactive TTR was localized in cells that were more centrally distributed in the islets, with an anatomic distribution that resembles that of the insulin-secreting β-cells. The presence of these retinoid-related proteins in the rat pancreatic islet suggested to these authors that retinoids and associated proteins have a metabolic role in the islets that in some manner supports the endocrine functions of the islets (20).
Nutritional approaches were also utilized by Chertow et al. in early studies to identify retinoid effects in islets present in the intact rat pancreas and in isolated rat islets studied in vitro (21). To assure complete retinoid-deficiency, weanling rats were maintained on a dietary regimen first described by Olson and colleagues (22,23). This protocol involved feeding of a totally retinoid-deficient but otherwise nutritionally complete diet for approximately 50 to 60 days followed by repeated cycling between a diet containing all-trans-retinoic acid (2 μg/g diet) and the totally retinoid-deficient diet. The authors reported that the retinoid-deficient rats gained less weight but maintained the same islet insulin content and the same islet cell size, number, and structure as rats fed a control diet. In vivo, retinoid-deficient rats displayed impaired glucose-induced acute insulin release and glucose intolerance. These effects were reversed upon repletion of the rats with retinyl palmitate but not upon repletion with all-trans-retinoic acid. Islets isolated from retinoid-deficient rats showed greatly diminished insulin secretion when challenged with glucose. Islets isolated from rats receiving 2 μg all-trans-retinoic acid/g diet showed the same impairment observed for the isolated retinoid-deficient islets. However, when rats were repleted with 8 μg all-trans-retinoic acid/g diet, insulin secretion by isolated islets was comparable to that of islets obtained from retinoid-sufficient rats (21). Chertow et al. reported that CRBPI protein levels were significantly decreased in isolated islets from retinoid-deficient compared to control-fed rats (21). This decline in CRBPI protein levels is in agreement with findings from Kato et al. (24) who had reported earlier that tissue levels of CRBPI protein decline in rats that had been maintained on a retinoid-deficient diet but not when the rats were maintained on a retinoid-deficient diet supplemented with all-trans-retinoic acid.
Subsequently, these same investigators also established that treatment of rats with retinyl palmitate protects β-cells from both streptozotocin- and alloxan-induced diabetes (25). The protective effects were dependent on the rout of retinyl palmitate administration. When retinyl palmitate was given intraperitoneally, the protective effect was greater than when given intravenously but when given by tail vein, the protective effect was dose-dependent.
In an independent study carried out some years later, Chertow et al. also explored the effects of retinoid-deficiency on glucagon secretion from rat pancreas and isolated islets (26). Arginine (19 mM) plus glucose (2.8 mM)-stimulated glucagon secretion was markedly impaired both in perfused pancreata and in perifused islets from retinoid-deficient rats. This finding held for perfused pancreata and perifused islets obtained from rats that had been repleted with retinoids for 2 to 4 weeks following their maintenance on the retinoid-deficient diet. However, consistent with their earlier studies, Chertow et al. reported that insulin secretion was normalized in isolated islets and pancreata obtained from the retinoid-repleted rats. These authors also reported that the hamster glucagon secreting α-cell In-R1-G9 cell line expresses both CRBPI and cellular retinoic acid-binding protein type I (CRABPI) [a cytosolic retinoic acid-binding protein (27)] and suggested that this cell line may be an appropriate one for studying retinoid effects on glucagon secretion (see below).
The insulin-secreting rat RINm5F cell line was used by Chertow and colleagues to study the biochemical effects of retinoids on β-cells (28-30). Initially, these investigators established, using radioimmunoassays and binding studies assessing [3H]retinol and all-trans-[3H]retinoic acid binding to proteins present in cytosolic extracts prepared from the cells, that the RINm5F cells express both CRBPI and CRABP1 (28). Later, they showed that treatment of cultured RINm5F cells with retinol (1.75 μM) or all-trans-retinoic acid (0.175 or 1.75 μM) increased KCl-induced insulin release (28). Subsequent Northern blot analyses established that the RINm5F cells expressed mRNA for RAR-α, -β, and -γ (29). When RARβ was overexpressed in RINm5F cells, treatment of transfected cells with all-trans-retinoic acid (0.1-1.0 μM) increased both cellular insulin content and insulin secretion compared to control cells (30). Interestingly, transfection of RINm5F cells with RARβ resulted in an inhibition of cell growth, with almost complete inhibition at all-trans-retinoic acid concentrations as low as 0.1 μM (31). Collectively, these data indicate that all-trans-retinoic acid acting through RARs has a significant role in maintaining/regulating normal insulin secretion from RINm5F β-cells (28-31).
Subsequent studies by these same investigators established an effect by the RXR pan-agonist 9-cis-retinoic acid on insulin secretion from RINm5F cells (30). [It should be noted that although 9-cis-retinoic acid was originally proposed to be a naturally occurring RXR ligand, this is now controversial due to the inability of many laboratories to detect this compound in tissues (10). However, 9-cis-retinoic acid is a strong RXR pan-agonist, albeit as a pharmacological one.] The RINm5F cells were found to express a single RXRα transcript and two RXRβ transcripts but no RXRγ transcripts (30). When RINm5F cells were cultured for 48 h in the presence of 9-cis-retinoic acid (0.001 to 1.0 μM) and then stimulated with glucose, 9-cis-retinoic acid-treatment increased insulin secretion by 50% to 100% over vehicle treated cells (30). Treatment of these cells with all-trans-retinoic acid employing the same conditions, increased glucose stimulated insulin secretion by 28% to 57%. When all-trans- and 9-cis-retinoic acid were added together to the cultured RINm5F cells, the combination was not additive or synergistic. Thus, these investigators proposed that 9-cis-retinoic acid, acting through RXR homodimers or heterodimers with RARs, stimulates insulin secretion from RINm5F cells (30).
Subsequent studies by Cabrera-Valladares et al. (32) independently confirmed that when isolated islets, prepared from either adult or fetal rats, were treated with retinoic acid (1.0 μM) for 24 hours resulted in an increase in glucose stimulated insulin secretion [the isomeric configuration of retinoic acid employed by these authors was not reported (32)]. Cabrera-Valladares et al. went on to demonstrate convincingly that retinoic acid treatment resulted in time-dependent increases in glucokinase (Gck) mRNA levels in both isolated adult and fetal islets and that this increase in fetal islets was associated with an increase in glucokinase promoter activity. A dose-dependent increase in glucokinase activity for both adult and fetal islets treated with retinoic acid was also observed. Other independent studies carried out by Blumentrath et al., employing the INS-1 β-cell line, confirmed that both all-trans- and 9-cis-retinoic acid treatments, when provided at 0.01, 0.1 or 1.0 μM for 48 h, increased insulin release from these cells (33). These authors further reported that RAR-α and -γ as well as RXR-α and -β proteins could be detected in extracts prepared from INS-1 cells. Both all-trans-retinoic acid treatment at 0.01, 0.1 or 1.0 μM for 48 h and 9-cis-retinoic acid treatment at 0.1 or 1.0 μM for 48 h resulted in an increase in glucose transporter type 2 (Glut2) mRNA expression in INS-1 cells. Moreover, both all-trans- and 9-cis-retinoic acid treatments (at 0.01, 0.1 or 1.0 μM for 48 h) inhibited INS-1 cell proliferation (33).
The effects of retinoid-treatment on glucagon secretion from isolated islets and glucagon-secreting cell lines were studied by Chertow and colleagues (34). These investigators employed intact isolated rat islets, hamster In-R1-G9 cells and mouse alpha TC-1 clone 9 tumor-derived glucagon-secreting cells in their studies. For intact islets, all-trans-retinoic acid treatment (0.01 or 0.1 μM) for 48 h inhibited glucagon secretion to approximately 60% that of control levels. Treatment of In-R1-G9 cells with retinol (0.175 to 5.0 μM) for 48 h inhibited glucagon secretion to 60% to 83% of control levels. All-trans-retinoic acid treatment (0.1 to 1.0 μM) for 48 h inhibited glucagon secretion to 72% and 43% of control levels for the In-R1-G9 cells. Treatment of alpha TC-1 clone 9 cells with retinol (1.75 μM) reduced glucagon secretion to 80% of control levels whereas all-trans-retinoic acid treatment (0.001 to 0.1 μM) for 48 h inhibited secretion from 83% to 68% of control levels. The inhibition of glucagon secretion from both In-R1-G9 and alpha TC-1 clone 9 cells by all-trans-retinoic acid was reported to be dose dependent. RARα transcripts were reported to be present in both cell lines and RARγ transcripts were also present in alpha TC-1 clone 9 cells. Thus, all-trans-retinoic acid, likely acting through its cognate RAR receptors, inhibits glucagon secretion from isolated rat islets and glucagon-secreting cell lines (34).
Recent advances in understanding retinoids and pancreatic islet and β-cell actions
The recent research focused on understanding retinoid actions in the islets of Langerhans have focused on the roles of 9-cis-retinoic acid and RXRs or all-trans-retinoic acid and RARs. Much of this research has involved the use of genetically manipulated mouse models and has primarily focused on β-cells and insulin synthesis and release.
Shimamura et al. reported data from a microarray study that showed that expression of aldehyde dehydrogenase 1A3 (Aldh1a3 or Raldh3), a gene encoding an enzyme that converts all-trans-retinaldehyde to all-trans-retinoic acid, was significantly increased in pancreatic islets obtained both from high fat diet-induced diabetic BDF1 mice and from diabetic C57BL/KsJ (db/db) mice (35). These authors further showed that exposure to culture medium containing a higher glucose concentration (25 mM) significantly increased Aldh1a3 expression in murine MIN6 and alpha TC-1 clone 9 cells, lines that were respectively derived from β- and α-cells of pancreatic islets. Overexpression of Aldh1a3 in MIN6 cells reduced insulin secretion, whereas overexpression of Aldh1a3 in alphaTC1clone 9 cells increased glucagon secretion from the cells. Moreover, knockdown of Aldh1a3 expression using siRNA decreased glucagon secretion from alpha TC1 clone 9 cells. Shimamura et al. proposed that increased expression of Aldh1a3 can dysregulate the normal balance between insulin and glucagon secretion in pancreatic islets, inducing β-cell impairments that lead to the development of type 2 diabetes (35).
Using a powerful genetic approach that employs an inducible dominant-negative form of RXRβ, one that ablates RXR transcriptional activity, Miyazaki et al. were able to show that the dominant-negative RXR enhanced insulin secretion upon stimulation with high glucose concentrations. This was taken to indicate that endogenous RXR negatively regulates glucose stimulated insulin secretion from β-cells (36). Using sophisticated molecular techniques, these authors were able to generate transgenic mice that specifically expressed a dominant-negative RXR-β transgene in β-cells but not in other cells. The transgenic mice expressing dominant-negative RXR-β showed elevated (improved) glucose tolerance and islets isolated from these transgenic mice also showed enhanced glucose-stimulated insulin secretion when manipulated in vitro. Interestingly, when Miyazaki et al. treated isolated islets obtained from the dominant-negative RXRβ transgenic mice and treated them with 9-cis-retinoic acid, they observed a significant decrease in glucose-stimulated insulin secretion. When plasma insulin levels were measured following an oral glucose challenge, these were significantly elevated 10 minutes after administration of glucose, by nearly 2-fold, in the induced transgenic mice compared to uninduced transgenic mice. Miyazaki et al. also reported a list of pancreatic genes whose expression was upregulated upon expression of the dominant-negative RXR-β transgene in a β-cell line. This listing included genes involved in regulating transcription, protein synthesis, signal transduction, protein trafficking and mitochondrial function. Although the mechanism underlying the inhibitory effect of RXR on glucose stimulated insulin secretion remains to be definitively established, the authors speculated that this may involve the actions of other transcription factors, specifically the peroxisome proliferator-activator receptors (PPARs), that are known to regulate transcription upon binding (heterodimerizing) to RXRs (36).
Simultaneous to the publication of the work by Miyazaki et al. (36), Napoli and colleagues reported that 9-cis-retinoic acid is present in pancreas and that its level decreases with feeding or after glucose dosing (37). Using liquid chromatography tandem mass spectrometry, these investigators reported that 9-cis-retinoic acid was present in the pancreas at concentrations ranging from 20 to 30 pmol/g tissue, a concentration that is well above those reported for pancreas all-trans-retinoic acid (37). 9-cis-retinoic acid was found to rapidly attenuate glucose sensing and insulin secretion. Pancreas 9-cis-retinoic acid levels were reported to vary inversely with serum insulin. These authors further established that 9-cis-retinoic acid treatment of isolated mouse islets in vitro attenuated glucose stimulated insulin secretion. When rat 832/13 β-cells were treated with 9-cis-retinoic acid, activity levels of Glut2 and Gck were reduced within 15 minutes, suggesting an effect of the retinoid on β-cell glucose sensing. After 2 hours of treatment, 9-cis-retinoic acid also reduced pancreatic and duodenal homeobox 1 (Pdx-1) and Hnf4α mRNA expression in 832/13 cells by approximately 8- and 80-fold respectively. When 832/13 β-cells were treated with the well studied synthetic RXR pan-agonist bexarotene and tested for glucose-stimulated insulin secretion, unlike for 9-cis-retinoic acid treatment, no decrease in insulin secretion was observed after 1 hour treatment of the cells with 23 mM glucose. However, bexarotene did reduce basal insulin secretion when stimulated with 3 mM glucose. Based on their data, Napoli and colleagues concluded that 9-cis-retinoic acid is a potent inhibitor of glucose-stimulated insulin secretion, likely acting in a non-genomic manner to modulate this process at high glucose concentrations (37).
In subsequent follow-up studies, Napoli and colleagues established that CRBPI modulates glucose homeostasis, at least partially through its regulation of pancreas 9-cis-retinoic acid levels (38). These studies showed that the pancreata of CrbpI-deficient mice have elevated concentrations of 9-cis-retinoic acid. This is associated with decreased expression of Glut2, Gck, and Pdx1 and decreased insulin and enhanced glucagon secretion in the fed state. Napoli and colleagues also noted that CrbpI-deficient mice are hyperglycemic, rely on increased fatty acid oxidation, and resist diet-induced obesity. These authors reported that in the CrbpI-deficient pancreas, mRNA levels of CrbpII, an intracellular binding protein that is normally present at high levels only in the intestine of adults (27), were elevated by approximately 80-fold. This is proposed to contribute to increased pancreatic 9-cis-retinoic acid synthesis from retinol and to account for the hyperglycemia observed in CrbpI-deficient mice. Thus, this work established that normal endocrine pancreas function, glucose homeostasis, and energy metabolism rely on CRBPI to modulate pancreas retinol metabolism and 9-cis-retinoic acid formation and levels (38).
Employing a genetic approach that is conceptionally similar to that of Miyazaki et al. (36), Brun et al. reported studies employing mice that expressed a dominant-negative RARα transgene whose expression was induced specifically in pancreatic β-cells in the adult (39). This strategy of inducing expression of the dominant-negative RARα transgene in adult β-cells rules out the possibility that transgene expression might adversely affect pancreas development in utero. The authors’ work established that all-trans-retinoic acid signaling mediated by RARs was required in the adult pancreas for maintaining both β-cell function and mass. Brun et al. reported that hypomorphism for RAR in β-cells led to a decrease in plasma insulin in the fed state and in response to a glucose challenge. As can be seen in Figure 2, glucose-stimulated insulin secretion was impaired in islets isolated from mice expressing the dominant-negative RARα transgene. This effect was mimicked by treatment of islets isolated from wild type mice with a RAR pan-antagonist. Treatment of wild type islets with either all-trans-retinoic acid or a RAR agonist increased glucose-stimulated insulin secretion. Among genes that are responsive to all-trans-retinoic acid, Glut2 and Gck mRNA levels were decreased in islets isolated from mice expressing the dominant-negative RARα transgene. Histologic analysis of pancreata expressing the dominant-negative RARα transgene revealed an approximate 50% decrease in both β-cell mass and insulin per β-cell, 1 month after induction of the dominant-negative transgene. The authors suggested that the observed decrease in β-cell mass in dominant-negative RARα expressing islets arose due to enhanced apoptotic loss of mature β-cells coupled with a diminished capacity to replenish β-cells when all-trans-retinoic acid/RAR signaling is disrupted.
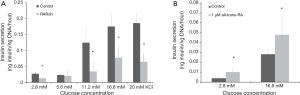
Interestingly, the dominant-negative RARα transgenic mice were reported to be euglycemic (39). This observation is consistent with the “glucagon hypothesis” of Unger and Cherrington (40) which proposes that glucagon is the proximal modulator of blood glucose in diabetes. Thus, the deficit in RAR signaling in β-cells of dominant-negative RARα transgenic mice was not enough to disrupt glucose homeostasis; whereas the Rbp1 knockout mice, which lack CRBPI in all tissues and cells, are hyperglycemic (38). Moreover, since CRBPI is reported to be expressed in α-cells of pancreatic islets (20) and Kane et al. (38) reported α-cell infiltration into islets and noted that this is a characteristic of glucagon hypersecretion, it would appear that retinoid metabolism in α-cells must be very important for regulating glucose homeostasis.
Simultaneously with the report by Brun et al. (39), Trasino et al. reported findings from studies of the essential actions of retinoids in pancreatic islets (41). These studies involved manipulating the dietary retinoid status of wild type and lecithin:retinol acyltransferase (LRAT)-deficient mice. LRAT is the predominant enzyme responsible for the synthesis of retinyl esters from retinol and consequently LRAT-deficient mice are unable to accumulate substantial retinoid stores (42,43). Thus, LRAT-deficient mice very quickly become retinoid-deficient when maintained on a retinoid-deficient diet (43). Trasino et al. reported data showing that all-trans-retinol is required for the maintenance of both pancreatic β-cell mass and for glucose stimulated insulin secretion in adult mice (41). Dietary retinoid deprivation was reported to cause greatly decreased pancreatic retinol levels, hyperglycemia, and reduced insulin secretion. Adult mice fed a retinoid-deficient diet for either 4 or 10 weeks were reported by Trasino et al. to display remodeling of the endocrine pancreas, marked β-cell apoptosis, shifts to a smaller islet size distribution, a 50% decrease in β-cell mass, increased α-cell mass, and hyperglucagonemia. Although the consumption of a retinoid-deficient diet could affect all retinoid-dependent tissues/cells in the body, the pancreatic β-cells were reported to be exquisitely sensitive to retinoid-deficiency associated apoptosis compared to other cells types in other tissues. These authors reported that retinoid-deficiency caused marked reductions in levels of CrbpI and the retinoic acid metabolizing enzyme cytochrome 26a1 (Cyp26a1) specifically in larger islets. Reintroduction of retinoid into the diet of retinoid-deficient mice restored pancreatic retinoid levels, glycemic control, normal islet size distribution, normal β-cell to α-cell ratios, endocrine hormone profiles and RARβ2 and RARγ2 transcript levels. Interestingly, reintroduction of retinoid into the diet did not involve increased β-cell proliferation or neogenesis.
Summary
It is clear from many published studies that all-trans-retinoic acid and RAR signaling are needed to maintain proper insulin secretion from pancreatic β-cells. It is also clear that retinoids also are needed to maintain β-cell mass within islets and to prevent β-cell apoptosis. However, as can be garnered from a reading of the above text, there are a number of very important unresolved issues regarding retinoid actions in pancreatic islets and how these may influence glucose homeostasis. The early literature suggests that 9-cis-retinoic acid can stimulate insulin secretion from β-cell lines whereas more recent evidence indicates that 9-cis-retinoic acid and RXRs independently act to dampen glucose stimulated insulin secretion. We are inclined to place more weight on the recent findings since the cell culture approaches used in earlier studies are more prone to experimental artifacts (for instance, artifactual isomerizationof 9-cis-retinoic acid to its all-trans-isomer). Another important issue in need of study concerns the effects of all-trans-retinoic acid on glucagon secretion from α-cells. Treatment of primary islet isolates with all-trans-retinoic acid is reported to result in decreased islet glucagon secretion; whereas overexpression of ALDH1a3, an enzyme that contributes to all-trans-retinoic acid synthesis, is reported to increase glucagon secretion. Thus, there is a need to resolve the role played by retinoids in regulating α-cell glucagon secretion. It is obvious to us that further research is needed in these areas before we are able to truly understand retinoid actions in maintaining glucose homeostasis.
Retinoids and pancreatic stellate cells (PSCs)
The liver stores the majority of retinoid that is present in the body. Specifically, HSCs store in their lipid droplets more than 50% of all the retinoid that is present in the entire body (12). However, other tissues also store retinoid as retinyl esters (12,13). It would appear, based on the literature, that the relatively well studied HSCs are a good cell model for understanding PSCs. The literature discussed below suggests that retinoid metabolism within PSC is very similar to that of HSCs. Thus, to facilitate understanding of retinoid metabolism in PSCs, we will first summarize briefly what is known regarding retinoid metabolism in HSCs. The retinoid found in HSC lipid droplets consists of long chain retinyl esters that account for more than 40% of the total lipid present in the lipid droplets (12). A micrograph showing the lipid droplets of freshly isolated mouse HSCs in culture is provided in Figure 3. Similar retinyl ester-containing lipid droplets are reported to be present in PSCs, but few biochemical details regarding these lipid droplets are available in the literature. It is well established that HSCs, upon activation following hepatic injury, contribute to the development of hepatic disease (44,45). This is the same as in the pancreas where PSC activation is understood to be a precursor for pancreatic disease. As the HSCs activate, they take on a myofibroblast-like phenotype and lose their lipid droplets and retinyl ester stores (12,44,45). Presumably, as PSCs activate they too loose retinoid stores but this is not definitively established in the literature. As noted below, as PSC activate and pancreatic disease ensues, many parameters associated with retinoid metabolism and actions have been reported to undergo changes.
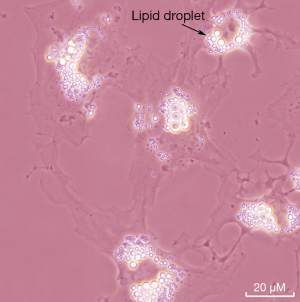
In healthy subjects, PSCs are quiescent and represent approximately 3-7% of the cell population, express desmin and glial fibrillary acidic protein, and possess cytoplasmic retinyl ester-containing lipid droplets (14,15). These cells possess long cytoplasmic processes and are located at the basolateral aspect of acinar cells (14). Exposure of PSCs to UV light at 328 nm elicits a transient blue-green fluorescence that is typical of the retinoid stores found in HSCs (15). However, little detailed biochemical information is available regarding the retinoid/lipid composition of PSC lipid droplets. The presence of retinoid-containing lipid droplets distinguishes PSCs from normal pancreatic fibroblasts. In culture, quiescent PSCs become activated, rapidly lose their retinoid-containing lipid droplets, take on a myofibroblast-like phenotype, and express α-smooth muscle actin (αSMA) and other markers of activation (14,15). Like HSCs in liver, upon injury to the pancreas, the PSCs become activated and synthesize excessive extracellular matrix proteins that contribute to pancreatic fibrosis and its sequelae (14,15). This is thought to be a first stage in the development of even more serious pancreatic disease that can culminate in pancreatic cancer (14,15). Although the loss of PSC retinoid stores is understood to be a component of disease development, this process is not as well studied as HSC activation and many mechanistic details are still missing.
The actions of retinoids in PSC activation has been studied by a number of laboratories. Jaster et al. established that cultured rat PSCs treated with all-trans-retinoic acid both proliferate at a slower rate and synthesize less collagen than vehicle treated control PSCs (46). This effect was reported to show dose dependence for all-trans-retinoic acid concentrations ranging from 0.1 to 10 μM. McCarroll et al. extended these findings by establishing that retinol, all-trans-retinoic acid or 9-cis-retinoic acid treatment results in reduced proliferation of culture-activated rat PSCs, as assessed by thymidine incorporation into cellular DNA as well as by direct cell count (47). This retinoid-dependent effect was associated with a significant decrease in activation of all three classes of mitogen activated protein kinase (MAPK) pathways and with increased RAR and RXR protein expression. These authors also reported that freshly isolated rat PSCs treated with retinol for five days showed significantly decreased expression of αSMA and fibronectin compared to controls (47). Moreover, McCarroll et al. showed that retinol supplementation prevents ethanol-induced activation and αSMA expression in freshly isolated rat PSCs.
Froeling et al. independently established that treatment of PSC lines in culture with all-trans-retinoic acid promotes a quiescent phenotype and altered expression patterns for genes involved in regulating cell proliferation, morphology and motility (48). These authors went on to demonstrate through analyses of an organotypic pancreatic ductal adenocarcinoma (PDAC) model, a genetically engineered mouse model for PDAC, and studies employing human samples that all-trans-retinoic acid treatment renders PSCs quiescent. Based on their extensive studies, Froeling et al. suggested a possible role for retinoids in blocking pancreatic disease associated with PSC activation (48).
Using retinoic acid response element (RARE-LacZ) transgenic reporter mice that mark cells with active retinoic acid signaling, Colvin et al. showed that in normal pancreas retinoic acid signaling was restricted to the islets of Langerhans and rare single, or small clusters of exocrine cells with an acinar or centro-acinar location and morphology (45). When Colvin et al. treated the reporter mice with cacrulein to induce pancreatic injury, they observed increased retinoic acid signaling in a large proportion of acinar cells immediately following cessation of cacrulein treatment. However, when the reporter mice were treated with 7,12-dimethylbenzanthracene to induce pancreatic cancer, to the contrary, a complete absence of retinoic acid signaling activity was observed in tumors or in precursor lesions despite analysis of multiple sections. Colvin et al. reported additional findings from study of tissue obtained from a cohort of 90 patients who had undergone pancreatic resection or biopsy. Immunohistochemical analysis of this tissue showed that CRBPI expression was significantly downregulated in 70% of these patients, with 50% of these having lost CRBPI protein expression completely. The loss of CRBPI expression was reported to be associated with reversible promoter hypermethylation (49).
The stroma of PDAC tissue is composed of relatively large amounts of extracellular matrix proteins, including collagen and hyaluronan, along with nerves, blood, and lymphatics, and a variable cellular population including inflammatory cells and activated PSCs (14,15). Making use of human tumor tissue and adjoining normal tissue obtained from PDAC patients and murine tumors obtained from the orthotopic Panc02 mouse model, Bleul et al. assessed tissue retinoid levels by highly sensitive high performance liquid chromatography mass spectrometry and gene expression profiles by real-time RT-PCR (50). These investigators reported that both all-trans-retinoic acid and all-trans-retinol levels were reduced in PDAC tissue compared to control tissue. Bleul et al. reported further that levels of the two retinoids were similarly reduced in pancreata obtained from the mouse model. Gene expression levels for genes encoding RARα, RARβ, RXRα and RXRβ were all found to be downregulated in PDAC tissue. Importantly, retention of expression of RARα or RXRβ was associated with a better overall survival of PDAC patients. Expression levels of the genes encoding CrbpI, Aldh1a1, an enzyme required for the formation of retinoic acid from retinol, and lecithin:retinol acyltransferase (Lrat), the enzyme responsible for the synthesis of retinyl esters from retinol, were all found to be downregulated in PDAC compared to normal pancreata (50).
Interestingly, Rovira et al. reported that they were able to purify, based on the presence of ALDH1A1 protein, a population of centro-acinar and terminal ductal progenitors from both the embryonic and the adult mouse pancreas (51). Since ALDH1A1 is involved in catalyzing the formation of all-trans-retinoic acid, the authors raise a question as to whether this enzyme and its action in retinoic acid synthesis has an important role in mediating the functions of these progenitors, or whether these cells serve as local sources for retinoic acid in a manner that organizes the surrounding cells (51).
Summary
The literature suggests an important role for retinoids and retinoid-related parameters (nuclear receptors and proteins involved in the metabolism of retinoids) in PSC activation and in pancreatic disease. This literature also raises the possibility that retinoids may be useful for preventing or slowing PSC activation and pancreatic disease progression. Although this is an attractive possibility, further research will be needed before the true validity of this proposal can be assessed in the clinic.
Role of retinoids in pancreatic islet and β-cell development in utero
The study of pancreatic development, and specifically the development of the endocrine pancreas, is an integral part of research aimed at developing new treatments for diabetes. For type 1 diabetes, at the present time insulin procurement is the only option. However, the success of islet transplantation has suggested that, as a therapeutic strategy, β-cell replacement may be a promising one. The paucity of available human pancreata for islet transplantation has put research aimed at developing new sources of functional β-cells at the forefront of diabetes research. Thus, it is very important to gain better understanding of pancreas development and islet/β-cell differentiation. Below, we will briefly consider the roles of retinoids, and particularly of all-trans retinoic acid, in pancreatic development and islet differentiation. This can be thought of as involving two stages: an early stage of pancreatic organogenesis and a later stage involving the specification of endocrine progenitor cells into β-cells and other islet cell types. For a more extensive information regarding the cellular and molecular processes that underlie endocrine pancreas development, the reader is referred to a number of extensive recent reviews (52,53).
All-trans-retinoic acid has a well studied essential role in patterning the development of large number of organs including the hindbrain, spinal cord, heart, eyes, forelimbs buds, lungs, genitourinary tract and the pancreas (54,55). Those actions of all-trans-retinoic acid are mediated through the activation of key target genes, predominantly by RARs and RXRs (54,55). Deficient or excessive retinoid-signaling arising either through dietary retinoid-insufficiency or through alterations of genes involved in retinoid metabolism or signaling can give rise to a wide spectrum of embryonic defects, emphasizing the importance of the regulation of intracellular retinoid forms and levels for assuring normal embryonic development.
In the early stages of pancreatic organogenesis, cells from the endoderm layer differentiate into pancreatic progenitor cells. Experimental studies that have blocked retinoic acid signaling early in embryogenesis through either pharmacological inhibition, mutations, or overexpression of a dominant-negative RARα transgene all agree that the ablation of retinoic acid signaling during early embryogenesis results in the loss of pancreatic endoderm differentiation. The retinoic acid required to maintain normal pancreatic endoderm differentiation is synthesized in the mesoderm and subsequently diffuses to the endoderm where it promotes the differentiation of endodermal cells into pancreatic progenitors. This involves the activation of key target genes, including the key regulatory gene Pdx1, which plays a critical role in the determination of pancreatic progenitors and pancreatic bud expansion (2,3). RAR signaling is required for maintaining pancreatic development and Pdx1 expression. Öström et al. reported that mice expressing the dominant-negative RARα transgene under the control of the Pdx1 promoter showed complete pancreatic agenesis, lacking both dorsal and ventral pancreas, and died in the neonatal stage (56).
The control of retinoic acid synthesis and degradation is an important mechanism for regulation of tissue differentiation. As can be seen from Figure 1, the synthesis and degradation of all-trans-retinoic acid are complex processes, involving many specific proteins and enzymes. Retinol dehydrogenase 10 (RDH10), an enzyme that catalyzes the conversion of retinol to retinaldehyde, plays a critical role in embryonic retinoic acid synthesis and organ development, including that of the pancreas (57,58). RDH10 has been shown to be indispensable for early organogenesis and synthesis of retinoic acid in the mesoderm that is required for differentiation of the endoderm germ layer cells into pancreatic progenitors (57). In the mouse, Rdh10 is first expressed in mesoderm around embryonic day (E) 8 (57). Rdh10 null mutations are reported to result in lethality by E10.5 to E14.5 (57). Notably, no pancreatic structures can be detected in Rdh10 null mutants. Those embryos display abnormalities characteristic of retinoic acid-deficiency and can be partially rescued by retinoic acid supplementation (57). Based on these data, it has been proposed that during embryogenesis Rdh10 gene expression in mesoderm plays a major role in the synthesis of retinoic acid that is required for the recruitment and differentiation of early pancreas progenitors.
A second enzymatic step needed for retinoic acid synthesis is the irreversible conversion of retinaldehyde to retinoic acid. ALDH1a2, expressed in the mesoderm, has been established to be responsible for retinoic acid synthesis at this stage of pancreas development. This is evidenced by the dorsal pancreas agenesis observed for Aldh1a2-null mutant mice (59,60).
Retinoic acid also acts essentially in the later stage of pancreas development to promote the generation of pancreatic endocrine progenitors and their differentiation into islets and β-cells (56). In the mouse, β-cells increase markedly in numbers from E14.5 onwards. Öström et al. reported that all-trans-retinoic acid promoted both the generation of Ngn3+ endocrine progenitor cells and their further differentiation into β-cells (56). These authors further established that Aldh1a1 is expressed in both mouse embryonic pancreas (for E13.5, E14.5, and E15.5) and human embryonic pancreas (from weeks 8 through 21) (56). The presence of ALDH1A1 protein in human embryonic pancreas was confirmed by Li et al. (61). Li et al. further established that the human embryonic pancreas also expresses ALDH1A2 and ALDH1A3 proteins (61). mRNAs for the three ALDH forms were expressed at relatively equal levels in 8-10 week human embryonic pancreata but expression of ALDH1a1 mRNA was elevated by about 2-fold by 20-21 weeks, whereas mRNA levels of ALDH1a2 and ALDH1a3 declined considerably (61). Pérez et al. reported findings from a study involving embryonic stem cells that they prepared from mice that were homozygous for a Rarβ-null mutation (62). The absence of Rarβ led to a decrease in terminal pancreatic differentiation and to a great reduction in expression of functional markers of islet function, including insulin and glucagon gene expression (62). Interestingly, Shen et al. reported that the addition of all-trans-retinoic acid to cultured mouse embryonic pancreas had distinct and separate effects on exocrine and endocrine cell differentiation (63). These authors reported that all-trans-retinoic acid treatment inhibited branching morphogenesis and exocrine cell differentiation while accelerating endocrine cell differentiation.
Summary
It is well established in the literature that all-trans-retinoic acid synthesized in the mesoderm diffuses to the endoderm where it promotes differentiation of endodermal cells into pancreatic progenitors. It is also well established that Rdh10 and Aldh1a2, which act to synthesize all-trans-retinoic acid are expressed in the mesoderm. The gene encoding the transcription factor PDX1, which is importantly involved in pancreatic differentiation, is directly activated by all-trans-retinoic acid. All-trans-retinoic acid, acting through RARs, acts in the later stages of endocrine pancreas development to promote the differentiation of endocrine progenitors into β-cells.
A number of very important questions regarding the role of retinoids in endocrine pancreas development remain to be established. For instance, are there genes that play important roles in pancreas development, other than Pdx1, that are induced or regulated by retinoids? Do retinoids have a role in influencing the differentiation of pancreatic islet cell types other than β-cells, most notably α-cells? Finally, given the research interest in differentiating embryonic stem (ES) cells for use in islet transplantation, it ultimately will be necessary to obtain precise understanding of the sequential activation of genes involved in retinoid metabolism during the differentiation of pancreatic progenitors into mature insulin secreting β-cells.
Acknowledgements
Funding: The authors would like to gratefully acknowledge support from the Thailand Research Fund (MRG5680074) (N Wongsiriroj), Mahidol University (N Wongsiriroj); and the U.S. Public Health Services, National Institutes of Health, grants R01 DK068437 (WS Blaner) and R21 AA021336 (WS Blaner).
Footnote
Conflicts of Interest: The authors have no conflicts of interest to declare.
References
- O’Byrne SM, Blaner WS. Retinol and retinyl esters: biochemistry and physiology. J Lipid Res 2013;54:1731-43. [PubMed]
- Gudas JM, Sporn MB, Roberts AB. Cellular biology and biochemistry of the retinoids. In: Sporn MB, Roberts AB, Goodman DS, editors. The Retinoids: Biology, Chemistry and Medicine. New York: Raven Press, 1994:443-520.
- Gudas LJ. Emerging roles for retinoids in regeneration and differentiation in normal and disease states. Biochim Biophys Acta 2012;1821:213-21.
- Palczewski K. Chemistry and Biology of Vision. J Biol Chem 2012;287:1612-19. [PubMed]
- Mangelsdorf DJ, Umesono K, Evans RM. The retinoid receptors. In: Sporn MB, Roberts AB, Goodman DS, editors. The Retinoids: Biology, Chemistry and Medicine. New York: Rave Press, 1994.
- Chambon P. A decade of molecular biology of retinoic acid receptors. FASEB J 1996;10:940-54. [PubMed]
- Chawla A, Repa JJ, Evans RM, et al. Nuclear receptors and lipid physiology: opening the X-files. Science 2001;294:1866-70. [PubMed]
- Balmer JE, Blomhoff R. Gene expression regulation by retinoic acid. J Lipid Res 2002;43:1773-808. [PubMed]
- Berry DC, Noy N. All-trans-retinoic acid represses obesity and insulin resistance by activating both peroxisome proliferation-activated receptor beta/delta and retinoic acid receptor. Mol Cell Biol 2009;29:3286-96. [PubMed]
- Al Tanoury Z, Piskunov A, Rochette-Egly C. Vitamin A and retinoid signaling: genomic and nongenomic effects. J Lipid Res 2013;54:1761-75. [PubMed]
- Brun PJ, Yang KJ, Lee SA, et al. Retinoids: Potent regulators of metabolism. Biofactors 2013;39:151-63. [PubMed]
- Blaner WS, O'Byrne SM, Wongsiriroj N, et al. Hepatic stellate cell lipid droplets: a specialized lipid droplet for retinoid storage. Biochim Biophys Acta 2009;1791:467-73.
- Senoo H, Yoshikawa K, Moril M, et al. Hepatic stellate cell (vitamin A-storing cell) and its relative--past, present and future. Cell Biol Int 2010;34:1247-72. [PubMed]
- Apte MV, Pirola RC, Wilson JS. Pancreatic stellate cells: a starring role in normal and diseased pancreas. Front Physiol 2012;3:344. [PubMed]
- McCarroll JA, Naim S, Sharbeen G, et al. Role of pancreatic stellate cells in chemoresistance in pancreatic cancer. Front Physiol 2014;5:141. [PubMed]
- Chertow BS, Baranetsky NG, Sivits WI, et al. Cellular mechanisms of insulin release. Effects of retinoids on rat islet cell-to-cell adhesion, reaggregation, and insulin release. Diabetes 1983;32:568-74. [PubMed]
- Soprano DR, Blaner WS. Plasma retinol-binding protein. In: Sporn MB, Roberts AB, Goodman DS, editors. The Retinoids: Biology, Chemistry and Medicine. New York: Raven Press, 1994:257-82.
- Li Y, Wongsiriroj N, Blaner WS. The multifaceted nature of retinoid transport and metabolism. Hepatobiliary Surg Nutr 2014;3:126-39. [PubMed]
- Napoli JL. Physiological insights into all-trans-retinoic acid biosynthesis. Biochim Biophys Acta 2012;1821:152-67.
- Kato M, Kato K, Blaner WS, et al. Plasma and cellular retinoid-binding proteins and transthyretin (prealbumin) are all localized in the islets of Langerhans in the rat. Proc Natl Acad Sci USA 1985;82:2488-92. [PubMed]
- Chertow BS, Blaner WS, Baranetsky NG, et al. Effects of vitamin A deficiency and repletion on rat insulin secretion in vivo and in vitro from isolated islets. J Clin Invest 1987;79:163-9. [PubMed]
- Lamb AJ, Piyaratana A, Olson JA. Induction of rapid, synchronous vitamin A-deficiency in the rat. J Nutr 1974;104:1140-48. [PubMed]
- Anzano MA, Lamb AJ, Olson JA. Growth appetite, sequence of pathological signs and survival following the induction of rapid, synchronous vitamin A-deficiency in the rat. J Nutr 1979;109:1419-31. [PubMed]
- Kato M, Blaner WS, Mertz JR, et al. Influence of retinoid nutritional status on cellular retinol- and cellular retinoic acid-binding protein concentrations in various rat tissues. J Biol Chem 1985;260:4832-38. [PubMed]
- Chertow BS, Webb MD, Leidy JW Jr, et al. Protective effects of retinyl palmitate on streptozotocin- and alloxan-induced beta cell toxicity and diabetes in the rat. Res Commun Chem Pathol Pharmacol 1989;63:27-44. [PubMed]
- Chertow BS, Driscoll HK, Blaner WS, et al. Effects of vitamin A deficiency and repletion on rat glucagon secretion. Pancreas 1994;9:475-84. [PubMed]
- Ong DE, Newcomer ME, Chytil F. Cellular retinoid-binding proteins. In: Sporn MB, Roberts AB, Goodman DS, editors. The Retinoids: Biology, Chemistry and Medicine. New York: Raven Press, 1994:283-318.
- Chertow BS, Moore MR, Blaner WS, et al. Cytoplasmic retinoid-binding proteins and retinoid effects on insulin release in RINm5F beta-cells. Diabetes 1989;38:1544-8. [PubMed]
- Chertow BS, Blaner WS, Rajan N, et al. Retinoic acid receptor, cytosolic retinol-binding and retinoic acid-binding protein mRNA transcripts and proteins in rat insulin-secreting cells. Diabetes 1993;42:1109-14. [PubMed]
- Chertow BS, Driscoll HK, Goking NQ, et al. Retinoid-X receptors and the effects of 9-cis retinoic acid on insulin secretion from RINm5F cells. Metabolism 1997;46:656-60. [PubMed]
- Chertow BS, Goking NQ, Driscoll HK, et al. Effects of all-trans-retinoic acid (ATRA) and retinoic acid receptor (RAR) expression on secretion, growth, and apoptosis of insulin-secreting RINm5F cells. Pancreas 1997;15:122-31. [PubMed]
- Cabrera-Valladares G, German MS, Matschinsky FM, et al. Effects of retinoic acid on glucokinase activity and gene expression and on insulin secretion in primary cultures of pancreatic islets. Endocrinology 1999;140:3091-96. [PubMed]
- Blumentrath J, Neye H, Verspohl EJ. Effects of retinoids and thiazolidinediones on proliferation, insulin release, insulin mRNA, GLUT2 transport protein and mRNA in INS-1 cells. Cell. Biochem Funct 2001;19:159-69. [PubMed]
- Chertow BS, Driscoll HK, Primerano DA, et al. Retinoic acid receptor transcripts and effects of retinol and retinoic acid on glucagon secretion from rat islets and glucagon-secreting cell lines. Metabolism 1996;45:300-5. [PubMed]
- Shimamura M, Karasawa H, Sakakibara S, et al. Raldh3 expression in diabetic islets reciprocally regulates secretion of insulin and glucagon from pancreatic islets. Biochem Biophys Res Commun 2010;401:79-84. [PubMed]
- Miyazaki S, Taniguchi H, Moritoh Y, et al. Nuclear hormone retinoid X receptor (RXR) negatively regulates the glucose-stimulated insulin secretion of pancreatic β-cells. Diabetes 2010;59:2854-61. [PubMed]
- Kane MA, Folias AE, Pingitore A, et al. Identification of 9-cis-retinoic acid as a pancreas-specific autocoid that attenuates glucose-stimulated insulin secretion. Proc Natl Acad Sci USA 2010;107:21884-89. [PubMed]
- Kane MA, Folias AE, Pignitore A, et al. CrbpI modulates glucose homeostasis and pancreas 9-cis-retinoic acid concentrations. Mol Cell Biol 2011;31:3277-85. [PubMed]
- Brun PJ, Grijalva A, Rausch R, et al. Retinoic acid receptor signaling is required to maintain glucose-stimulated insulin secretion and β-cell mass. FASEB J 2015;29:671-83. [PubMed]
- Unger RH, Cherrington AD. Glucagonocentric restructuring of diabetes: a pathophysiologic and therapeutic makeover. J Clin Invest 2012;122:4-12. [PubMed]
- Trasino SE, Benoit YD, Gudas LJ. Vitamin A deficiency causes hyperglycermi and loss of pancreatic β-cell mass. J Biol Chem 2015;290:1456-73. [PubMed]
- O’Byrne SM, Wongsiriroj N, Libien J, et al. Retinoid absorption and storage is impaired in mice lacking Lecithin:Retinol Acyltransferase. J Biol Chem 2005;280:35647-57. [PubMed]
- Liu L, Gudas LJ. Disruption of the lecithin:retinol acyltransferase gene makes mice more susceptible to vitamin A deficiency. J Biol Chem 2005;280:40226-34. [PubMed]
- Geerts A. History, heterogeneity, developmental biology, and functions of quiescent hepatic stellate cells. Semin Liver Dis 2001;21:311-35. [PubMed]
- Friedman SL. Hepatic Stellate Cells: Protean, Multifunctional, and Enigmatic Cells of the Liver. Physiol Rev 2008;88:125-72. [PubMed]
- Jaster R, Hilgendorf I, Fitzner B, et al. Regulation of pancreatic stellate cell function in vitro: biological and molecular effects of all-trans-retinoic acid. Biochem Pharmacol 2003;66:633-41. [PubMed]
- McCarroll JA, Phillips PA, Santucci N, et al. Vitamin A inhibits pancreatic stellate cell activation: Implications for treatment of pancreatic fibrosis. Gut 2006;55:79-89. [PubMed]
- Froeling FE, Feig C, Chelala C, et al. Retinoic acid-induced pancreatic stellate cell quiescence reduces paracrine Wnt-β-catenin signaling to slow tumor progression. Gastroenterology 2011;141:1486-97. [PubMed]
- Colvin EK, Susanto JM, Kench JG, et al. Retinoid signaling in pancreatic cancer, injury and regeneration. PLoS One 2011;6:e29075. [PubMed]
- Bleul T, Rühl R, Bulashevska S, et al. Reduced retinoids and retinoid receptors' expression in pancreatic cancer: A link to patient survival. Mol Carcinog 2015;54:870-9. [PubMed]
- Rovira M, Scott S-G, Liss AS, et al. Isolation and characterization of centroacinar terminal ductal progenitor cells in adult mouse pancreas. Proc Natl Acad Sci USA 2010;107:75-80. [PubMed]
- Shih HP, Wang A, Sander M. Pancreas organogenesis: From lineage determination to morphogenesis. Annu Rev Cell Dev Biol 2013;29:81-105. [PubMed]
- Cano DA, Soria B, Martin F, et al. Transcription control of mammalian pancreas organogenesis. Cell Mol Life Sci 2014;71:2383-402. [PubMed]
- Duester G. Retinoic acid synthesis and signaling during early organogenesis. Cell 2008;134:921-31. [PubMed]
- Rhinn M, Dollé P. Retinoic acid signalling during development. Development 2012;139:843-58. [PubMed]
- Oström M, Loffler KA, Edfalk S, et al. Retinoic acid promotes the generation of pancreatic endocrine progenitor cells and their further differentiation into β-cells. PLoS One 2008;3:e2841. [PubMed]
- Sandell LL, Sanderson BW, Moiseyev G, et al. RDH10 is essential for synthesis of embryonic retinoic acid and is required for limb, craniofacial, and organ development. Genes Dev 2007;21:1113-24. [PubMed]
- Rhinn M, Schuhbaur B, Niederreither K, et al. Involvement of retinol dehydrogenase 10 in embryonic patterning and rescue of its loss of function by maternal retinaldehyde treatment. Proc Natl Acad Sci USA 2011;108:16687-92. [PubMed]
- Molotkov A, Molotkova N, Duester G. Retinoic acid generated by Raldh2 in mesoderm is required for mouse dorsal endodermal pancreas development. Dev Dyn 2005;232:950-7. [PubMed]
- Martín M, Gallego-Llamas V, Ribes M, et al. Dorsal pancreas agenesis in retinoic acid-deficient Raldh2 mutant mice. Dev Biol 2005;284:399-411. [PubMed]
- Li J, Xhi C, Yeung FS-H, et al. Aldehyde dehydrogenase 1 activity in the developing human pancreas modulates retinoic acid signalling in mediating islet differentiation and survival. Diabetologia 2014;57:754-64. [PubMed]
- Pérez RJ, Benoit YD, Gudas LJ. Deletion of retinoic acid receptor β (RARβ) impairs pancreatic endocrine differentiation. Exp Cell Res 2013;319:2196-204. [PubMed]
- Shen C-N, Margurie A, Chien C-Y, et al. All-trans retinoic acid suppresses exocrine differentiation and branching morphogenesis in the embryonic pancreas. Differentiation 2007;75:62-74. [PubMed]